Architected materials for impact absorption: A complete guide

Written by nTop
Published on September 21, 2022
Architected materials and additive manufacturing unlock new opportunities for managing impact absorption. You can maximize your control over the collapse with the right tools and design methodology.
When it comes to absorbing an impact, something has to give. Generally, there is one of two things collapsing: a protective structure like a helmet or the sensitive object protected by the helmet (e.g., a person’s body or head). Fortunately, engineers have some influence over the former, tailoring the structure’s deformation to absorb and reduce the impact energy. This level of design control is rapidly expanding with technologies such as additive manufacturing. Architected materials, in particular, offer a vast design space to tune and optimize this deformation capability and the resulting energy absorption capacity. This post will explore some design principles to help you optimize architected materials for impact protection.
Watch the presentation below on architected materials for impact absorption to learn more.
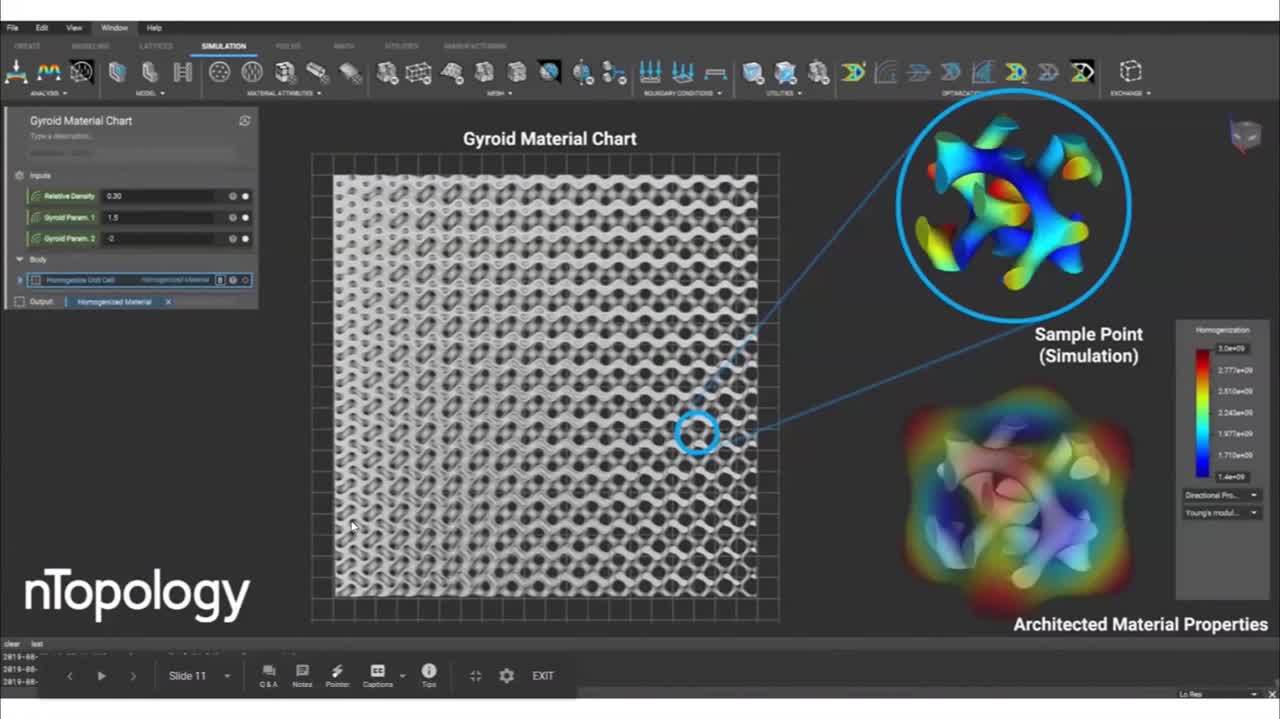
Screenshot from video presentation on architected materials for impact absorption.
What Are Architected Materials?
Architected materials are highly-engineered structures with tailored mechanical, thermal, electromagnetic, or biological performance characteristics. They can have programmable properties that can vary in space or over time — even in combinations not found in nature.
When paired with additive manufacturing, architected materials unlock new opportunities for advanced product design. These opportunities include everything from 3D printed foams for impact absorption to porous structures that promote bone growth and from soft robotics to exotic metamaterials.
Architected Materials Applications
There are many potential uses for architected materials across various industries and applications, including aeronautical, automotive, biomechanical, and more.
Because architected materials can absorb high amounts of mechanical energy, they are suited to applications for which crashworthiness and the storage and diffusion of energy are important. For example, lattice structures are regularly used for packaging purposes and in protective equipment, like helmets.

This helmet design concept features a conformal lattice structure with a custom unit cell to increase impact absorption.
Architected materials also show a high degree of promise in regenerative medicine. The pores and channels within the structure allow for the diffusion of blood and nutrients, which is essential for the regeneration process. Additionally, the high porosity and surface area to volume ratio provide the necessary conditions for positioning growth factors in the biomaterials volume.
Depending on the objective of the architected material, architecting specific material properties can be approached with an ordered pattern, a stochastic pattern, close-cell, or an open-cell. In the case of a close-cell approach, air is often inside the cells, which can be relevant for impact absorption as this air creates a cushioning effect.
Manufacturing Architected Materials
In the last decade, there have been significant advancements in manufacturing and fabrication methods. These developments have made it possible to produce complex structures for architected materials using various additive manufacturing methods.
- Light-based processes such as stereolithography (SLA), digital light processing (DLP), and other variations of the vat photopolymerization process are among the most flexible methods for adding material to build a complex structure.
- Extrusion-based fabrication processes, such as fused deposition modeling (FDM), are also well suited to fabricate architected materials of low-to-medium complexity and at a lower cost.
- Polymer and metal powder-bed fusion technics, like Selective laser sintering (SLS), multi-jet fusion (MJF), selective laser melting (SLM), and electron beam melting (EBM), have also been used to fabricate high-performance architected materials.
Each of these methods has its own advantages and disadvantages, but all hold the potential to produce high-quality architected materials. As the technology continues to evolve, even more methods will likely be developed, giving designers a greater number of options for creating innovative products.
Architected Material Properties
There are endless combinations of geometries and materials you can print from with architected materials, so the design challenge can quickly become overwhelming. The well-known Ashby chart shown below provides a good representation of the objective of architected materials.

Ashby chart showing the strength (MPa) of various materials related to Young’s modulus, E (GPa), and the architected materials profile.
The chart represents all materials available to engineers — from foams to woods to plastics and complex alloys. The purpose of architected materials is to close the white space on this chart. For example, say you can’t print in wood, but you need to mimic the properties of wood. Architected materials can allow you to print in an alternative material while replicating wood’s properties.
Nature is always finding new ways to optimize materials to support its purpose. Though slow in this optimization, nature is highly effective at multi-functional design. Take bone, wood, and honeycomb as an example. These materials don’t just offer structural benefits but also facilitate fluid storage or transfer. This combination of properties best represents the ultimate goal of architected materials — to do more with less, often by introducing multiple functions.
Anisotropic Properties: Going with the Grain
Anisotropy refers to objects or materials with a physical property that has a different value when measured in different directions. Optimizing these anisotropic materials is difficult with conventional tools like topology optimization, which generally considers isotropic material (having a physical property with the same value when measured in different directions).
Just as woods and fiber composites have a distinct grain or bias to them and resultant directional properties, so do architected materials — which are very rarely isotropic. In woods, the difference in compressive strength when oriented along or against the grain can differ significantly. Fiber composites allow you to control properties in-plane by strategically orienting the fabric layers. However, they require you to design the overall structures to avoid out-of-plane loading, where they tend to delaminate.

Directional properties in a few different classes of materials, with blue arrows loosely indicating relative directional strengths. Wood and composites are two fairly well-understood material classes where engineers utilize anisotropy to their advantage. Architected materials offer the ultimate control in anisotropy, but this design freedom makes optimization complex.
Additively manufactured lattices, however, are the ultimate in customization potential for directional properties — the only limitations being the manufacturing process, design tools, and your imagination.
A single unit cell can not only be tuned to morph its material properties as desired (see diagram below) but spatially varied through the part to locally tailor to your specifications. The opportunities are endless, so where do you even begin?
Designing Lattices For Impact Absorption
The ability to freely tune material properties in 3D allows you to architect your material properties to fit your application. With enough unit cells in the lattice, one can treat the structure as a new material. Below is a visual representation of this on a unit-cell level. The grey is the physical unit cell to be manufactured, and the colored shape indicates its directional material properties.

Various unit cells and a visualization of their corresponding effective directional stiffness properties. The two honeycombs are oriented in different directions and show a stark difference in directional properties along their ‘grain’ or principal axis. These structures exhibit high inertial sensitivity when dynamically loaded along these directions.
It is assumed that quasi-static loading will be applied to the material in this design process. However, under impact conditions, the properties of the material may change. Many base materials are strain-rate sensitive and exhibit different properties based on the loading rate.
When designing a product, you have probably already down-selected a few materials that can be 3D printed. This fact allows you to focus the design decisions on the architecture. In a similar line of thinking, you have probably selected a temperature range, too. Focusing primarily on the architecture allows an apples-to-apples comparison and can get us surprisingly far.
Modes of Energy Dissipation
When designing lattices for impact absorption, we must consider energy dissipation. For example, consider a football player wearing a helmet upon impact with another player. The energy from that impact must be dissipated either through the helmet or the protected object — which, in this case, is the football player’s head.
Structures generally deform to absorb energy, either elastically (it absorbs the impact and then springs back) or plastically (it remains deformed and is only suitable for one-time use). The latter is common in vehicles, which deform plastically in the event of a crash.
Even the lightest lattices cannot escape Newton’s Laws of Motion. Every strut or element in the architected material has inertia and will remain at rest unless a force acts upon it. The intricate arrangements of material in these structures can make a spring-like maze for forces and shocks to traverse and contributes to what is known as the inertial sensitivity of the structure.
Calladine and English — researchers at Cambridge University — first described these effects as “strain-rate” and “inertia effects” that are related respectively to the material and topology characteristics of the structure.

A summary of the strain rate and inertial sensitivity work conducted by Calladine and English. The two classes of structures identified by their quasi-static compressive response perform very differently when the loading rate increases.
Notice the force-displacement and energy-displacement (i.e., the integral) response for the two types of structures pictured above.
- Type II has a strong start and absorbs energy early but softens rapidly as the structure buckles and collapses.
- Type I is weaker at first but maintains this steady plateau throughout the collapse.
Calladine and English dropped heavy weights on their specimens. Upon applying the same energy, the curved Type I structure crushed significantly more than the kinked Type II, with the straighter walls of the latter putting up more inertial resistance to the impact load. The reason for this is rather intuitive: generally, the more material aligned with the direction of loading, the more mass there is to redirect (or inertia) to crush it.
Impact Absorption & AM Lattice Structures
As demonstrated in the chart below, these theories also apply to additively manufactured materials. With the right unit cell, the degree of Type I or Type II behavior can be freely varied based on aligning this material with the loading direction.

Quasi-static compression of a few different parameterized unit cells, which vary in their material alignment, showing the tunability between Type I and II responses. The more folded structures behave as a soft and steady-collapsing Type I structure, while the more prismatic honeycomb-like structures are much stronger at first but lose much of this strength as they collapse.
Inertial sensitivity means that the structure will perform differently under impact conditions. Indeed, the strength, energy absorption, and even deformation mechanisms can vary significantly compared to quasi-static compression.
However, as alluded to earlier, the quasi-static response can get us surprisingly far, even in making predictions in the dynamic world. The force-deflection response can often be used to predict whether a structure is Type I or Type II and thus whether strain-rate or inertial effects will be dominant.
Often, with a stiff and strong unit cell architecture, you will find a more inertially sensitive structure. With a softer, more foam-like configuration, inertia is less of a factor, and strain-rate sensitivity of the material may be more of a consideration. Of course, this behavior is also anisotropic.
You can see in the image below how this can extend to architected materials and how it might even vary within the same structure.

Spatially varying architected materials, where anisotropy and predicted inertial sensitivity are varied through the structure along the axes, as indicated by arrows. Compare these unit cell geometries to the schematics of Type I and II structures shown in the previous diagram.
Where to begin?

A helmet liner concept based on an origami-inspired architected material.
Many more factors are at play in designing protective structures: from physics (material strain-rate sensitivity, fatigue over repeated impact cycles, off-angle threat protection, blunt impact vs. penetration resistance, etc.) to human factors (helmet fit, environmental conditions, or if it is even worn).
However, equipped with the right tools, you can have substantial control over the anisotropy and inertial sensitivity in architected materials and use these characteristics to their advantage in designing their parts. Understanding these two factors will build the foundation for optimizing the rest.
When designing for impact absorption, begin by asking yourself the following questions:
- How much energy do you need to absorb?
- What is the stress limit for what you are protecting?
- Which unit cells can best achieve this statically?
- How do they change dynamically?
- Does the material matter?
Lastly, accept and prepare for the reality that designing with architected materials inherently requires many iterations to optimize. Fortunately, the latest advanced engineering design software facilitates endless iteration and optimization with maximum control.
Key takeaways
- Architected materials are highly-engineered structures with tailored mechanical, thermal, electromagnetic, or biological performance characteristics.
- Additively manufactured lattices are the ultimate in customization potential for directional properties — the only limitations being the manufacturing process, design tools, and your imagination.
- Additive manufacturing allows you to unlock the potential of architected materials, but you need the right design software to manage this newfound complexity. nTop gives you tools to generate, control, and fine-tune complex lattice structures with engineered properties.
nTop
nTop (formerly nTopology) was founded in 2015 with the belief that engineers’ ability to innovate shouldn’t be limited by their design software. Built on proprietary technologies that upend the constraints of traditional CAD software while integrating seamlessly into existing processes, nTop allows designers in every industry to create complex geometries, optimize instantaneously, and automate workflows to develop breakthrough parts and systems in record time.
Related content
- CASE STUDY
Replacing spacecraft supermaterial with high-performance lattice
- VIDEO
Creating a computational design workflow to lightweight drone panels with nTop’s new ribbing tools
- VIDEO
nTop Siemens Energy at CDFAM NYC 2024
- CASE STUDY
Reducing weight to help win and withstand the rigors of a 24 hour Le Mans race
- VIDEO
Design better implants for osseointegration with the Lattice Pore Size Block